
New directions for brain tumor research emerge at the intersection of cancer and neuroscience

UCSF neurosurgeon Shawn Hervey-Jumper leads cutting-edge interdisciplinary program
When Tom Paquin was diagnosed with glioblastoma in March 2021, he was struck by how little had changed about the treatment and prognosis in the years since his father had died of the same disease. As a tech innovator who co-founded Netscape and later Mozilla, he wanted to see research that would shift the way scientists are thinking about this type of cancer.
In conversations with neurosurgeon Shawn Hervey-Jumper, MD, Paquin and his family learned about preclinical studies over the last seven years focusing on the microenvironment of glial tumors and how these tumors co-opt cellular and network responses in the brain to their benefit.
This emerging body of research offers a new framework for scientists – one where the previously siloed fields of brain tumor biology and neuroscience overlap. And Hervey-Jumper, as director of the UC San Francisco Glial Tumor Neuroscience Program (GTNP), is building research infrastructure complete with the recruiting, training, and clinical development pipelines to develop new approaches for treating gliomas.
The inaugural GTNP Symposium, held earlier this year in October, marked the beginning of this interdisciplinary effort generously supported by the Paquin family and offered the scientists themselves an opportunity to communicate with each other about burgeoning collaborations.
Talks given at the symposium also gave a window into how a modern understanding of neuroscience might change some of what we know about how brain tumors form and grow, as well as offer some radically different ideas about potential treatments and cognitive rehabilitation strategies.
Glial Tumor Formation
For years, researchers studying gliomas in mouse models suspected that the tumors may arise from precursor cells that would normally become the glial cells that support the brain’s electrical circuits. Glial cells called oligodendrocytes add myelin to the axons of neurons to speed neurotransmission throughout an individual’s life, and some glial tumor cells even look like oligodendrocyte precursor cells at the molecular level. Many brain tumor cells also develop from cells resembling neural stem cells.
But the developing human brain has more types of neural stem cells than a mouse brain, the predominant experimental model for preclinical research. Neural development is also a much lengthier process in humans than in mice. The extended time that human neural progenitor cells spend growing and dividing helps contribute to the larger number of neurons and other brain cells. But scientists say these periods may also create new vulnerabilities when mutations and injuries could have a big impact on the brain.
“In order to develop better preclinical models, we really need to develop human-based models,” said Tomasz Nowakowski, PhD, an assistant professor in the departments of neurological surgery and anatomy, at the GTNP symposium.
Nowakowski and his colleagues are turning to stem cells made from human skin cells that have been reverted to an earlier developmental state. Scientists can then subject these stem cells to the same instructions they would normally receive to form various types of brain cells. These neural stem cells grown in dish can also self-organize into three-dimensional structures, or brain organoids, that resemble many of the early stages of brain development.
Researchers can also introduce specific genetic mutations, like disease-relevant or cancer driver mutations, into the stem cells to study their effect on brain development, Nowakowski said. For example, Mercedes Paredes, MD, PhD, an associate professor in the Department of Neurology, and her colleagues are using brain organoids to model tuberous sclerosis, a multi-system genetic disease that results in benign brain tumors called subependymal giant-cell astrocytomas as well as seizures and cognitive disabilities.
Brain organoids are still missing two important types of cells found in brain tumor microenvironment: microglial and endothelial cells. But Nowakowski’s research group is looking into ways to reintroduce these cells into the organoids. Their work so far shows that brain organoids with microglia develop more synchronized patterns of spontaneous electrical activity than those without. These results, Nowakowski said, suggest that scientist can more accurately model the more complex network properties of the brain by better recapitulating the various cell types in the developing brain.
Hervey-Jumper and Nowakowski are collaborating to derive brain organoids directly from patient brain tumor samples in addition to stem cells. Galina Popova, PhD, a postdoctoral scholar in Nowakowski’s lab, is also fusing these patient tumor samples with brain organoids as a model for studying CNS metastasis.
Other researchers like new neurosurgery faculty member Cathryn Cadwell, MD, PhD are conducting detailed studies of human brain cell types and circuits at the level of single cells. Her collaboration with Hervey-Jumper as well as Edward Chang, MD, and Kurtis Auguste, MD, involves using brain samples from patients who have undergone functional brain mapping. Her research team is taking that tissue directly from the operating room and recording the electrical activity of the neurons.
Cadwell is examining these patient samples using classical histologic techniques to establish the diagnosis, the tumor grade, and tumor cellularity. Then, she performs next-generation sequencing assays at the level of single brain cells.
Incorporating all this information, Cadwell said, provides a more holistic understanding of how brain circuits work in people as well as how disease alters those functions.
These new experimental approaches to studying the brain using patient samples will help scientists understand brain tumors in a more clinically relevant way. The findings could also help generate novel therapies for glioblastoma that overcome the challenges posed by the high degree of variation between cells within the tumor and across different patients.
Tumor Growth
“Brain tumors don’t just grow and live in the brain on their own,” Hervey-Jumper said. “They live in this complex environment where electrochemical interactions influence their growth.”
For example, a large subpopulation of glioblastoma cells forms connections with other tumor cells and astrocytes through structures called tumor microtubules that help the tumor evade chemotherapy and surgery.
Additionally, in a recent paper published in Cell, researchers at the University of Heidelberg, showed that a subpopulation of glioblastoma cells not connected to other glial cells called astrocytes or other tumor cells are the ones driving the tumor’s invasion into other parts of the brain. These cells – which share molecular features with neuronal and oligodendrocyte precursor cells – move into noncancerous brain tissue much like how new neurons in the developing brain migrate out to their appropriate location.
The glial tumors themselves are also electrically active tissue, and their electrical activity drives tumor growth. The synapses between the glioblastoma cells and neurons can facilitate the continued formation of the tumor microtubules that promote infiltration as well as the speed with which the tumors cells invade other healthy tissue.
Gliomas also restructure the circuits in the brain. Studies in mice had demonstrated that glioma cells increase the excitability of neighboring neurons and in doing so, remodel the circuitry in the brain. But scientists were not sure whether the same phenomenon was occurring in humans until Hervey-Jumper and Stanford neuro-oncologist Michelle Monje, MD, PhD, began collaborating.
Hervey-Jumper interrogated the cortical surface of glioblastoma using intraoperative electrocorticography. In these studies, they confirmed the fact that gliomas reorganize rather than destroy neurons. In fact, these electrical recordings showed that areas of the cortex that have been infiltrated by the tumor exhibit neuronal hyperexcitability.
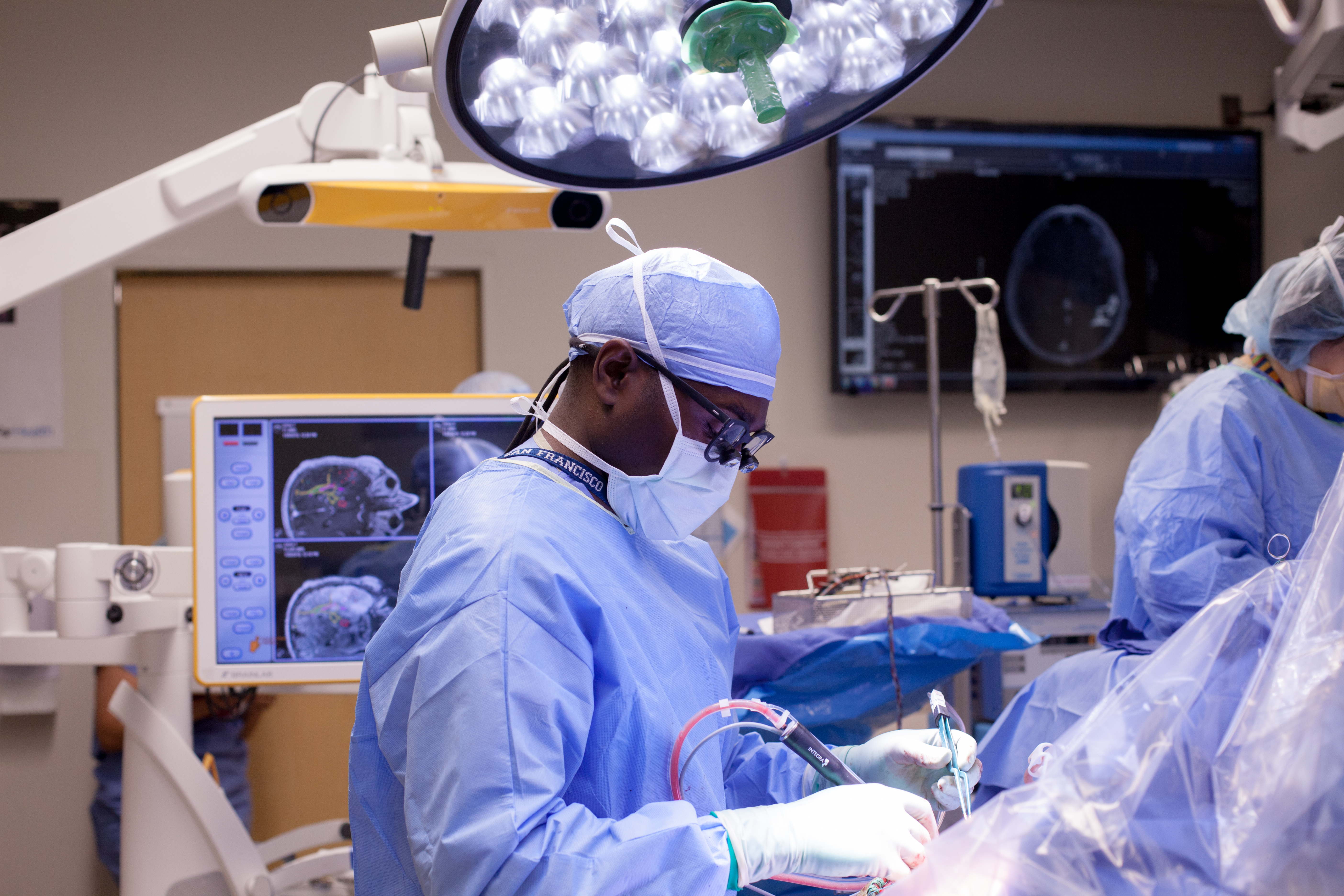
Improving Patient Outcomes
In collaboration with UCSF radiology professor Srikantan Nagarajan, PhD, and his colleagues in the Biomagnetic Imaging Laboratory, Hervey-Jumper is also examining how different regions of the brain interact and coordinate their electrical activity using a noninvasive neuroimaging technique called magnetoencephalography (MEG). The scientists can look at how coordinated neural oscillations in one area of the brain are connected with others within specific frequencies – a measure of functional connectivity called synchrony.
Tumors that are more synchronous with other parts of the brain are more integrated into the brain network. People with brain tumors that have less synchronous activity with the rest of the brain have better outcomes. These glioblastoma patients tend to both live longer and do better in language cognition tests.
The emerging research on how gliomas reshape brain networks may help scientists like neuropsychologist Christina Weyer-Jamora, PhD, RN, and neuro-oncologist Jennie Taylor, MD, MPH, figure out how to tailor cognitive rehabilitation strategies to better address the impairments in attention, learning and memory, and executive function that many patients with glioma face.
Hervey-Jumper and his colleagues have also been comparing regions that have low and high functional connectivity in patient biopsies at the level of single-cell gene expression. In their preliminary studies, the researchers see that genes responsible for helping neural circuits form are more abundant in tumor regions with higher functional connectivity and that a protein called TSP-1 helps maintain the connections between glioblastoma cells and neurons. Levels of this protein in patient blood samples also correlates with the degree of connectivity between glioblastoma and healthy regions of the brain, suggesting the protein could serve as a clinical biomarker.
Modulating the bidirectional communication between glial tumor cells and neurons presents a new avenue for effective cancer therapies. One strategy would be to use a drug that would inhibit electrical activity in the brain to slow brain tumor growth. Under the Tom Paquin Brain Tumor Research project, Hervey-Jumper and his research team are testing strategies to treat glioblastoma cells with a drug that blocks the secreted protein TSP-1 to inhibit neuronal activity dependent tumor growth.
This view of glioma growth on a network level also invites consideration of existing neuromodulation devices that affect electrical signaling. Scientists could potentially electrically stimulate certain parts of the brain to modulate tumor growth or enhance neuronal recovery.
These devices could also be used to enhance cognitive function by bypassing the parts of the brain damaged by either the tumor or the persistent neuroinflammation resulting from chemotherapy and radiation. For example, Hervey-Jumper’s research group has demonstrated that although glioma-infiltrated cortex has a lower capacity for encoding speech information, it recruits a broader network of cortical regions. This finding could indicate how different regions of the cortex can compensate for injury.
By integrating fresh perspectives and new technology from both developmental neurobiology and systems neuroscience, the GTNP is ready to make big strides in understanding the impact of malignant glioma and treatment on brain function, and opportunities for novel therapies.
Support the cutting-edge research of the Glial Tumor Neuroscience Program here: makeagift.ucsf.edu/GTNP